Recent Research
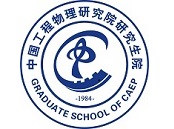
Please feel free to contact us if you have any questions or you want to know more about our reserach.
§ Highly nonlinear light-nucleus interaction
The interaction between light and atomic nuclei is typically weak and confined within the linear and perturbative regime, which limits the achievable nuclear excitation probability and impedes potential applications such as nuclear optical clocks, nuclear lasers, and nuclear energy storage. In this work we show that the interaction between hydrogenlike thorium-229 ions (229Th89+) and contemporary intense lasers propels light-nucleus interaction into a highly nonlinear and nonperturbative regime. This interaction unlocks remarkably efficient nuclear excitation: over 10% of the 229Th nuclei can be excited to the isomeric state by a single femtosecond laser pulse. Moreover, the laser-driven 229Th89+ ions radiate multiple wavelengths of light that are high-order harmonics of the laser frequency, akin to the high harmonic generation process from laser-driven atoms but with distinct characteristics. These results pioneer a new frontier for exploring light-matter interaction, furnish a powerful method for efficient control over atomic nuclei, and pave a new way of nuclear coherent light emission.
Reference: [1] H. Zhang, T. Li, and X. Wang, Phys. Rev. Lett. 133, 152503 (2024).
§ Nuclear hyperfine mixing effect: Nucleus in an electron "resonant cavity"
Nuclear physics and atomic physics generally focus on different aspects of physics: the former primarily on nuclear states, nuclear reactions, and nuclear transitions, while the latter mainly on electron states and electron transitions. However, the nucleus and electrons can also interact with each other. One significant process is called the nuclear hyperfine mixing (NHM) effect. Electrons, especially inner-shell electrons, can generate a strong electromagnetic field at the nucleus, causing mixing between different nuclear states and altering the properties of the nucleus. For example, the lifetime of the first excited state of the 229Th nucleus is approximately 1000 seconds, but if there is a 1s electron, this excited state’s lifetime can be reduced by five orders of magnitude to about 0.01 seconds. Research on the NHM effect has almost exclusively focused on the 229Th nucleus, because the energy of its first excited state is very low (only about 8 eV), making it relatively easy to mix with the ground state. However, in our recent work [1], we proposed a new mechanism, greatly expanding the application scope of the NHM effect. Unlike the known hyperfine mixing mechanism, which uses only one electron state (such as the 1s electron state in the case of 229Th), this new mechanism utilizes two electron states. If the energy difference between these two electron states matches the nuclear level difference (with angular momentum matching as well), a significant NHM effect may occur. For instance, we predict that in the boron-like lead-205 ion (205Pb77+), the lifetime of the first nuclear excited state could be reduced by four orders of magnitude from 15 minutes to 32 milliseconds due to this effect. The energy of the first excited state of the 205Pb nucleus is 2329 eV higher than the ground state. Before this new mechanism was proposed, the community did not expect this nucleus to exhibit a significant NHM effect. However, due to the presence of a pair of electron states (2p1/2 and 2p3/2 states) with energy (2356 eV) close to the nuclear energy and matching angular momentum, significant NHM is predicted to occur for the first time at the keV level of nuclear energy. This new mechanism is similar to an atom in an optical cavity, as shown in the figure above. A two-level atom in an optical cavity has dressed states that are the system’s eigenstates, where the atomic states are coupled with the field states. Similarly, a two-level nucleus in an electron "resonant cavity" (i.e., an ion with suitable electronic states) has dressed states that are the system’s eigenstates, where the nuclear states are coupled with the electron states, leading to the NHM effect. The modulation of the lifetime of the nuclear excited state by the electron cavity is also similar to the modulation of the spontaneous emission lifetime of an atomic excited state by controlling the light-field mode in an optical cavity. The NHM effect greatly enhances the coupling between light and the nucleus. The significant shortening of the lifetime of the nuclear excited state is a result of this effect enhancing the coupling between the nucleus and the vacuum light field. Conversely, this effect also correspondingly increases the efficiency of laser excitation of the nucleus, which is of great significance for research in laser nuclear physics. Laser nuclear physics is an emerging interdisciplinary field that combines advanced laser light sources such as high-intensity lasers and X-ray free-electron lasers with nuclear physics. It represents a new frontier in the study of light-matter interactions and has been receiving increasing attention in recent years.
Reference: [1] W. Wang and X. Wang, Phys. Rev. Lett. 133, 032501 (2024).
§ 229Th nuclear physics and strong-field atomic physics
Efficient excitation of 229Th from the nuclear ground state to the 8-eV isomeric state remains a challenging problem to be solved. In our recent publication [1], we consider isomeric excitation of 229Th in a laser-heated cluster. A 229Th cluster is first radiated by an intense femtosecond laser pulse, causing ionization of the constituting atoms. The cluster will then survive for a time on the order of 1 ps, during which the electrons collide with the nuclei repeatedly and excite them to the isomeric state. Two mechanisms are responsible for the isomeric excitation: nuclear excitation by electron capture (NEEC) and nuclear excitation by inelastic electron scattering (NEIES). Other mechanisms, including nuclear excitation by bound-bound electronic transitions and photoexcitations, are estimated to be at least several orders of magnitude weaker and can be neglected. Our calculation shows that for relatively weak laser intensities around 1014 W/cm2, the excitation mechanism is dominantly NEEC. And for relatively strong laser intensities around 1016 W/cm2, the excitation mechanism is dominantly NEIES. One can tune between NEEC and NEIES continuously by changing the laser intensity. This laser-heated-cluster scheme not only provides an efficient means for isomeric excitation, but also provides an approach for the confirmation of the NEEC process. The NEEC process has been predicted theoretically for nearly half a century [2], but it has not been confirmed conclusively in experiments. Recently, two beam-based experiments aim to confirm NEEC with 93mMo, but contradicting results are reported [3, 4]. Laser-cluster interaction provides a laser-based, in contrast to the more traditional beam-based, approach for the experimental verification of the NEEC process.
References: [1] J. Qi, H. Zhang, and X. Wang, Phys. Rev. Lett. 130, 112501 (2023). [2] V. I. Goldanskii and V. A. Namiot, Phys. Lett. B 62, 393 (1976). [3] C. J. Chiara et al., Nature 554, 216 (2018). [4] S. Guo et al., Phys. Rev. Lett. 128, 242502 (2022).
§ 229Th nuclear physics and strong-field atomic physics
Among all known nuclei the 229Th nucleus has a unique low-lying isomeric state with an energy of 8 eV above the nuclear ground state. The existence of this isomeric state has fascinated the scientific community with potential applications of a "nuclear clock" or a "nuclear battery". How to excite the 229Th nucleus from the ground state to the isomeric state in a controllable and efficient way remains an open question and is also the first step towards the fascinating applications. We propose an excitation approach based on laser-driven electron recollision, as illustrated in the above figure. Recollision is the core process of strong-field atomic physics. It has been well understood that the recolliding electron may (i) recombine radiatively to the ion core leading to high harmonic generation; or (ii) kick out another electron leading to nonsequential double ionization; or (iii) elastically scattered by the ion core leading to laser-induced electron diffraction. With the 229Th nucleus, the recolliding electron has a new channel of exciting the nucleus. It is interesting to see the connection between strong-field atomic physics and nuclear physics with the 229Th system. Details can be found in our recent publication [1,2].
References: [1] W. Wang, J. Zhou, B. Liu, and X. Wang, Phys. Rev. Lett. 127, 052501 (2021). [2] X. Wang, Phys. Rev. C 106, 024606 (2022).
|